This is a plain-language summary of “Connecting Fuel Cell Catalyst Nanostructure and Accessibility Using Quantitative Cryo-STEM Tomography”, an open-access (free) article in the Journal of the Electrochemical Society
You’ve probably heard the proverb: “If you want to go quickly, go alone. If you want to go far, go together.” Metaphorically, this is sound advice, but literally many of us today are used to going both far and fast regularly thanks to our cars and other modern vehicles.
The ability to travel both far and fast is convenient, but also impressive from the standpoint of basic physics. To travel far you need to be able to bring a large amount of energy to keep you going, and to travel fast you need to be able to use a large amount of power, which physicists define as the rate at which you use energy (power = energy / time).
Conventional modern cars can travel both far and fast by burning gasoline in internal combustion engines. Gasoline stores a huge amount of energy and doesn’t weigh too much, making it a very energy-dense fuel, and modern combustion engines have been masterfully engineered to deliver large amounts of power.
Unfortunately, gasoline-burning combustion engines have some major drawbacks. Burning gasoline and other fossil fuels creates pollution, including carbon dioxide, which causes climate change, as well as soot particles and chemicals that harm our health and the environment. To make things worse, combustion engines are very inefficient. The goal of the engine is to turn the chemical energy in the fuel into motion (mechanical energy), but they do this by first burning the fuel to make heat, and inevitably most of the energy is lost as the heat dissipates before it can be used.
Electric cars provide us with a promising solution to the downsides of gasoline-burning cars. They don’t emit any pollution and use energy very efficiently. However, scientists and engineers are still working on making sure that they can go both as far and as fast as the cars that we are used to.
There are two general types of electric cars: battery electric vehicles (BEVs) and fuel cell electric vehicles (FCEVs). Both types work by converting chemical energy directly into electricity, without an inefficient “burning” step, which powers an electric motor to move the car. In battery electric vehicles, (like the Nissan Leaf, Chevy Bolt, and Tesla’s cars,) the battery both stores the chemical energy and converts it into electricity. In FCEVs (like the Toyota Mirai and Hyundai Nexo), the chemical energy is stored as hydrogen fuel, typically in a high-pressure tank, and the fuel cell converts it into electricity by reacting it with oxygen in the air. A BEV is re-charged by plugging it in, similar to a cell phone or laptop, while a FCEV is re-filled at a hydrogen station, similar to refilling a gasoline car at a gas station.
BEVs have no trouble with power, and some are spectacularly fast, but they can struggle to store enough energy to go very far, which is a problem for interstate road trips or hauling freight. Getting more range means adding more batteries, which gets expensive and heavy. FCEVs don’t have a problem going far, since adding more range just requires a bigger hydrogen tank, adding comparatively little cost and weight. The hard part is making the fuel cell provide enough power to make the car fast at a reasonable cost.
To make electric power, fuel cells split hydrogen into protons and electrons on one side and combines them with oxygen on the other to make water. The fuel cell powers a circuit (like a car motor) by separating these two sides with a membrane that lets protons pass through but blocks electrons so that they are forced to travel across by pushing through the circuit.
Making these reactions happen fast takes some tricks. The especially hard part is combining the protons and electrons with oxygen molecules. To get them all close enough together to react, they need to stick on the surface of a metal, called the catalyst. A good catalyst has to solve a Goldilocks problem: we need things to stick to it strongly enough to react, but not so strongly that they won’t come off afterward. The best catalysts for this reaction are made with platinum, which is unfortunately very expensive. But a little bit of platinum can go a long way if used carefully. The key reactions happen on the platinum surface, and you can get a huge surface area out of a small amount of platinum by making it into nanoparticles that are only a few atoms wide. (A couple grams – about the weight of a peanut – of platinum nanoparticles can have the same surface area as a tennis court.) Platinum nanoparticles used for fuel cell catalysts are typically stuck onto nano-sized carbon (which is basically fancy soot), called the carbon support, to keep them from clumping up, transport electricity, and to leave space for water and air to move around them. A gooey, proton-conducting material called ionomer is mixed in as well to allow the protons to reach the platinum.
For the reaction to happen quickly, these different materials all need to be arranged just right so that the protons, electrons, and oxygen can all get to the catalyst surface easily. If any one of them can’t arrive fast enough, the reaction will stall and the fuel cell won’t be able to provide any more power for the car.
My co-workers and I wanted to find out how the shape of the carbon support affects the pathways for water and oxygen to get to the catalyst so that we can squeeze as much power as possible out of our precious platinum. We tried two different types of carbon supports – one that is solid so that all of the platinum nanoparticles sit on its surface, and one that is full of holes, like a sponge, so that the platinum nanoparticles sit both on the outside of the carbon and inside the holes. We took 3D images of the catalyst particles using an electron microscope to study the sizes and shapes of the platinum nanoparticles and carbon supports, and to see how much of the platinum is inside or outside of the carbon support.
By comparing our 3D images of the catalyst on different carbons to their performance in real fuel cells, we learned about how the reactants moved around each type of carbon and how this gave strengths and weaknesses to each type of carbon. We found that with a solid carbon support it was easy to get protons and oxygen to the platinum surface, since it was all exposed on the outside of the carbon, but the ionomer covers up the platinum surface and makes it hard for the oxygen and protons to react on it. When the platinum nanoparticles were inside the holes in the carbon they didn’t get covered in ionomer, so the surface was more effective as a catalyst. We discovered that protons were still able to get to the platinum inside the holes as long as we kept everything wet enough so that the holes were full of water. However, the holes were so small that they made it difficult for oxygen to get to the platinum inside the carbon fast enough, which limited the amount of power that the fuel cell could provide.
After learning about the details of how the reactants moved around on these carbon supports, our coworkers were able to design a better carbon support that had bigger holes in the carbon to let oxygen get inside more easily while still keeping out the ionomer that could gunk up the platinum surface. When they built fuel cells using their new carbon support they were able to get almost twice as much power from the same amount of platinum on the old carbon supports.
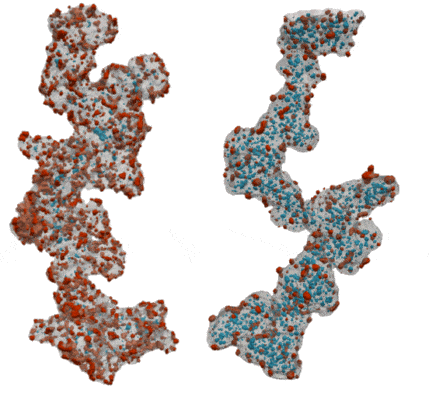